Challenges and Opportunities when Powering Automotive SoC Core Rails

Get valuable resources straight to your inbox - sent out once per month
We value your privacy
Introduction
The automotive industry is undergoing a major transformation to support electric vehicles (EVs), autonomous driving, high-end infotainment systems, vehicle connectivity, and software-defined vehicles. The goals and benefits of EV transformation, and its impact on the automotive supply chain, are well understood by now.
However, the digital transformation towards autonomous driving and high-performance computing (HPC) is in the early stages and still evolving. This transformation applies to both EVs and internal combustion engine (ICE) vehicles. The technology disruption due to these up-and-coming trends will create challenges and opportunities for automotive supply chains.
Due to continued population growth and urbanization, modern transportation suffers from congestion, accidents, and lack of access to mobility, which have adverse socioeconomic effects (see Figure 1). The creation of autonomous and connected vehicles for personal use and robotaxis for public transportation will improve convenience, safety, and economic mobility.
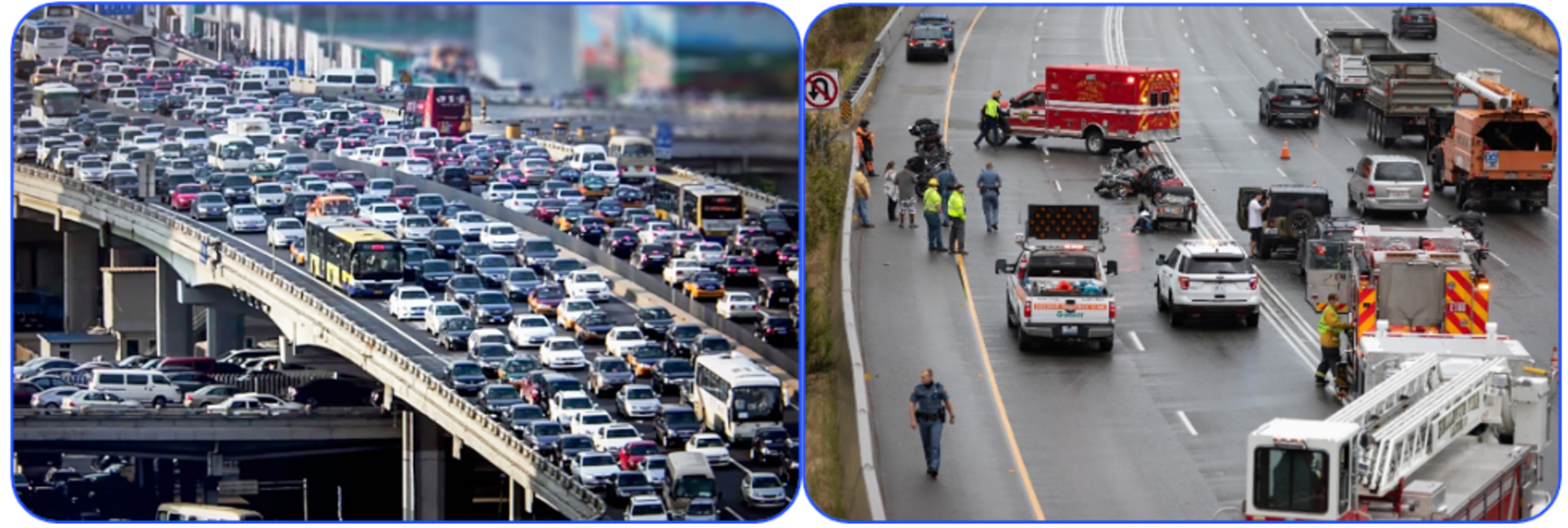
Figure 1: Issues when Driving
The increasing need to digitize vehicles requires advanced technology, new architectures, and the development of innovative components and solutions across the board. Autonomous vehicles require a tremendous amount of high-performance computational horsepower for not only the vehicle itself, but also for the supporting infrastructure.
To enable digitized mobility, automotive original equipment manufacturers (OEMs) and Tier 1s are partnering with system-on-chip (SoC) suppliers to provide the computational power required to advance the automotive industry. This paradigm shift is an opportunity for manufacturers to create new functions and features to satisfy the needs of the modern consumers, as well as to differentiate their products from competitors.
The Evolution of Automotive Computing
Figure 2 shows architecture evolution of automotive computing.
In the past, tens of electronic control units (ECUs) distributed across the vehicle were used to process localized data. Today, domain controllers aggregate and process data for a section of the vehicle. The vehicle of the future will have a central computer to provide the required computational horsepower to support advanced driver assistance features, high-end infotainment systems, connectivity, and other state-of-the-art features.
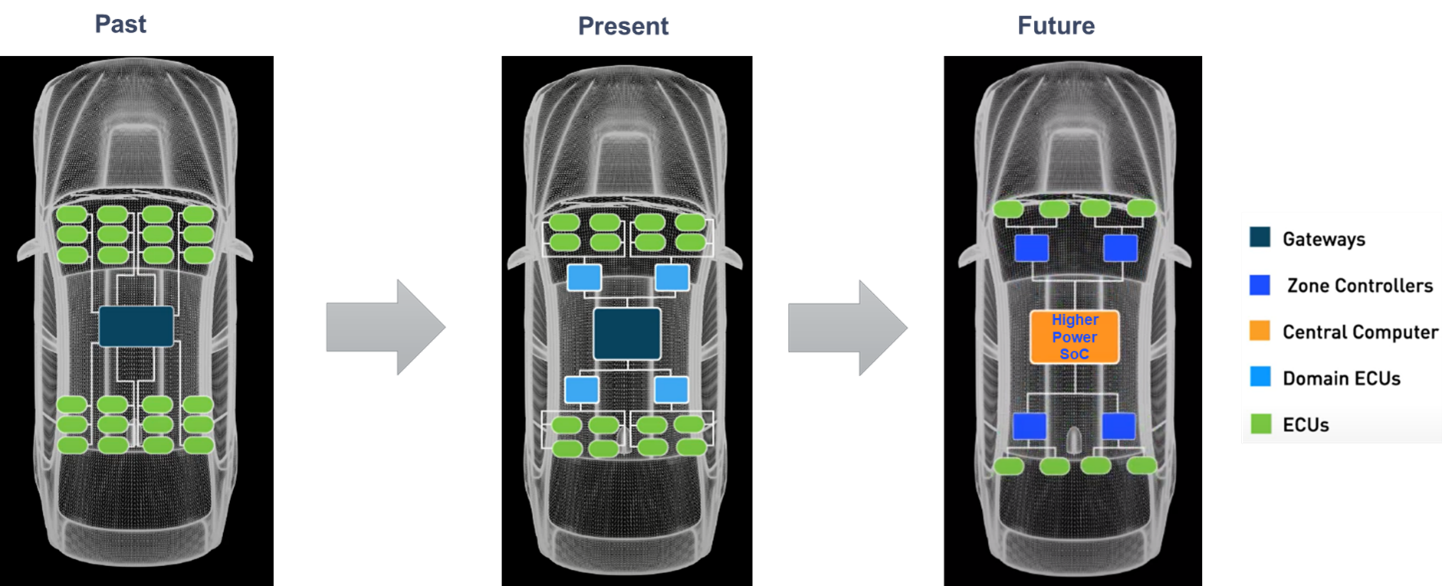
Figure 2: Evolution of Automotive Computing
The central computers for future vehicles will be run by powerful SoCs. These SoCs have advanced CPU and GPU capabilities that process large amounts of data and perform the complex computations that allow vehicles to make decisions in real time. These SoCs require advanced power management solutions, specifically for the core voltage rails.
SoC core rails require hundreds of amperes of current, with stringent transient performance and efficiency requirements. Servers, datacenters, and AI applications have used powerful SoCs and advanced power management solutions for a few generations already, but these solutions are new for automotive applications. Automotive power solutions have additional challenges, such as providing AEC-Q100 qualification and ASIL-D functional safety while maintaining the same high efficiency, fast transient response, configurability, scalability, monitoring, and system protection features as the enterprise SoC core power solutions.
Automotive Systems-On-Chip (SoCs)
Figure 3 shows a simplified power tree for automotive SoCs, divided into high-power core rails and low-power system rails. The low-power rails can use power management ICs (PMICs) or discrete point-of-load (PoL) converters. The high-power core rails require specialized solutions because they have stringent specifications to provide the power needed by the CPUs and GPUs embedded into the SoC. In addition, the SoC may require more than one core rail, depending on its architecture and performance specifications. This article will focus on power solutions for the SoC core rails.
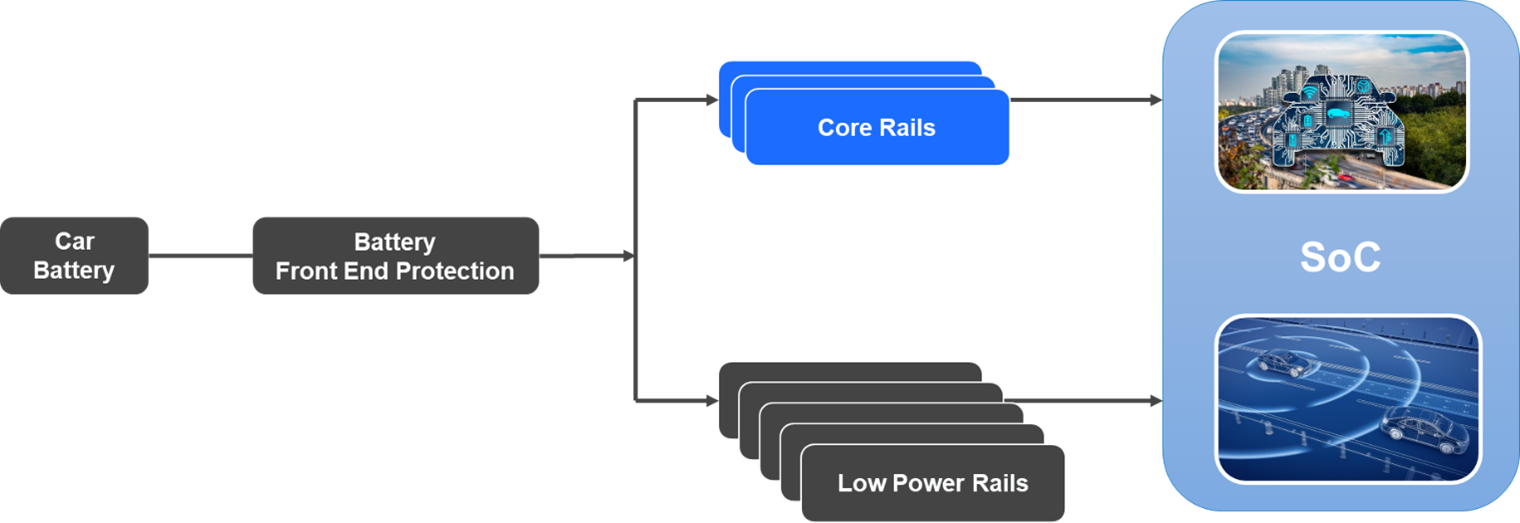
Figure 3: Typical Power Tree of an Automotive SoC
Traditional solutions for SoC core rails use analog pulse-width modulation (PWM) controllers, discrete MOSFETs, and discrete current- and temperature-sensing circuitry (see Figure 4). These solutions require many external components, which raises costs, reduces reliability for automotive applications, and requires a larger PCB area. This can make traditional solutions difficult to design and susceptible to a lack of flexibility and scalability, which is a critical requirement for the types of SoCs used in advanced driver assistance systems (ADAS), infotainment, and high-performance computing (HPC) applications.
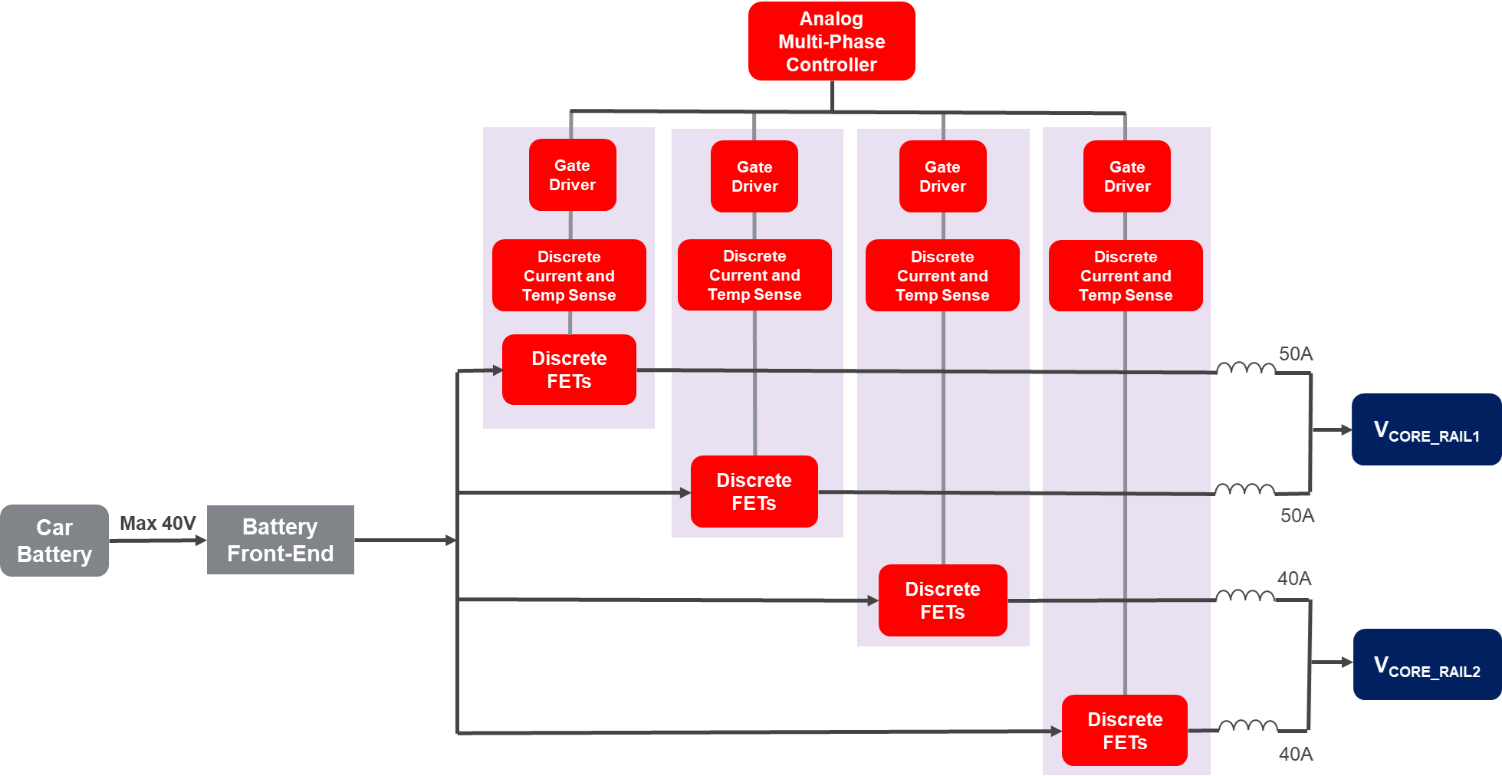
Figure 4: Traditional SoC Solution
Figure 5 shows a state-of-the-art SoC core power solution using digital multi-phase controllers and monolithic DrMOS power stages. The DrMOS integrates the gate driver IC, current-sensing circuit, and temperature-sensing circuit; this enables a simpler solution by eliminating several external components required by traditional solutions.
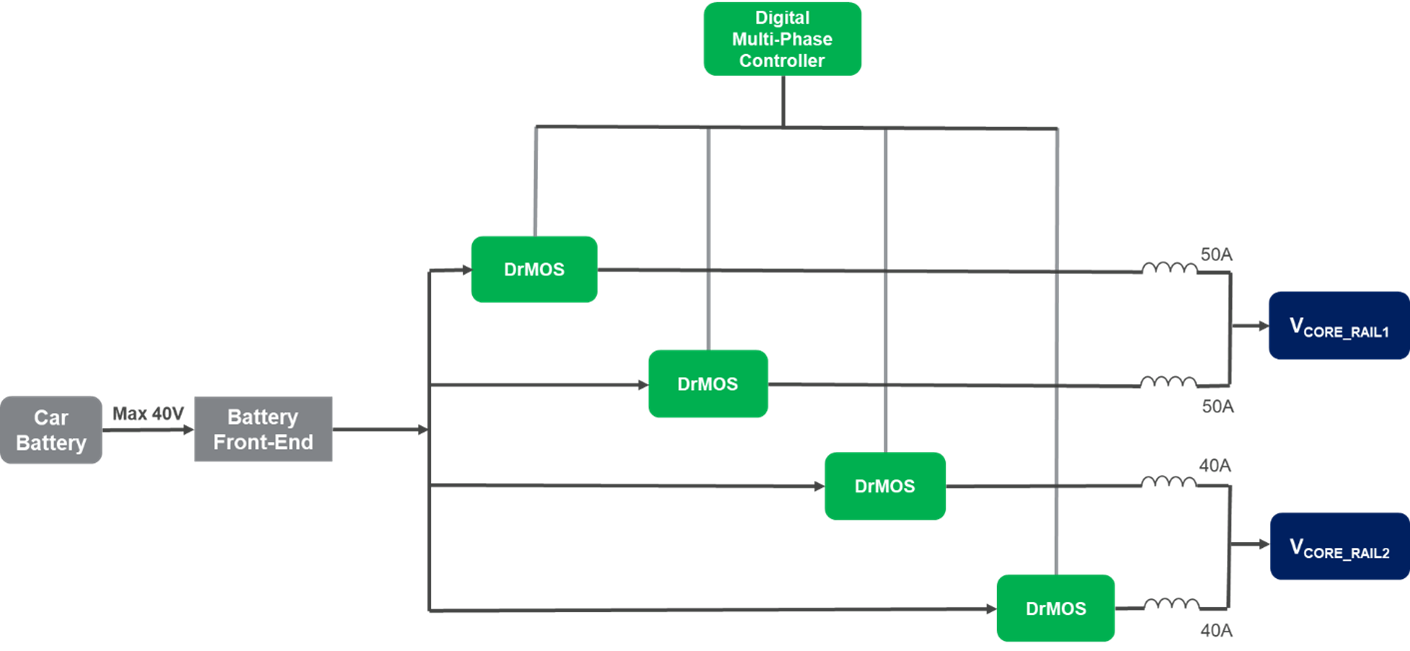
Figure 5: Digital PWM Controllers and Monolithic DrMOS
The DrMOS is a monolithic design, which offers incredibly high power density, accurate current-sensing, and accurate on-die temperature sensing. MPS has 22V and 6V DrMOS portfolios to support single-stage power conversion and two-stage power conversion.
These digital controllers offer flexibility and scalability since the number of phases can be configured depending on the current ratings of the given SoC core rail. Digital controllers do not require any external feedback loop compensation, which simplifies design work and cuts down on development time. They also feature a non-volatile memory (NVM) to configure and reconfigure the register settings up to 1,000 times. Finally, the controller and DrMOS offer various monitoring and protection features that can be used to implement system-level telemetry.
Automotive SoC and Batteries
Modern automotive vehicles have two options for the 12V battery: a lead-acid battery, or a lithium-ion battery. Lithium-ion batteries have a maximum output voltage (VOUT) up to 20V. Lead-acid batteries have a maximum 40V VOUT during transients.
Figure 6 shows a single-stage power conversion implementation using 22V-rated DrMOS products. The vehicles that use a lithium-ion 12V battery can use the 22V DrMOS directly off the battery without the need for a pre-regulator to convert the battery voltage down to SoC core rail voltages. This is an ideal implementation for best efficiency, smaller PCB area, lower cost, and optimized electrical performance.
For vehicles that use a lead-acid battery, the maximum voltage can reach up to 40V during load dump or double battery conditions. In this scenario, a pre-regulator is used to limit the input voltage (VIN) on the DrMOS to a maximum of 20V in order to protect the DrMOS during transients.
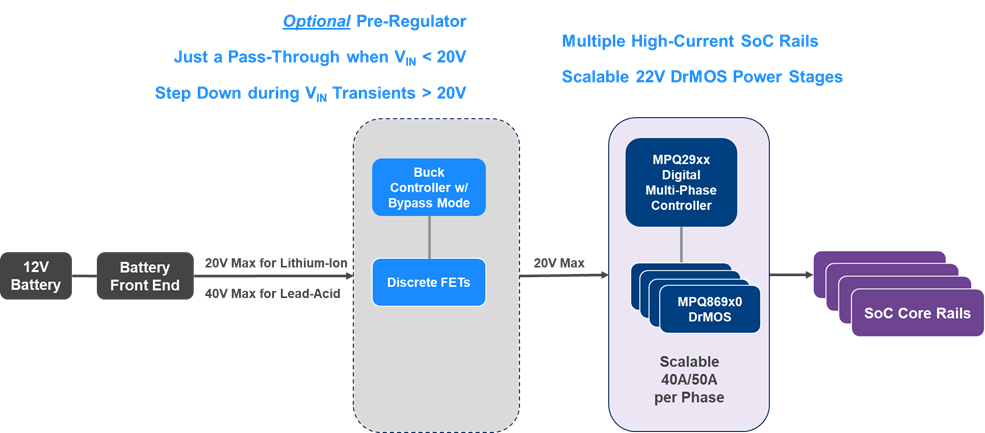
Figure 6: Single-Stage Power Conversion using 22V-Rated DrMOS with Optional Pre-Regulator
If the vehicle has a lead-acid battery, a pre-regulator can be used as the voltage limiter. This pre-regulator can operate at a 100% duty cycle ratio, which means that under normal operating conditions, it is simply a pass-through providing >99% efficiency. The pre-regulator works as a buck converter during voltage transients to limit the DrMOS’s VIN to 20V for a few milliseconds when the battery voltage (VBATT) exceeds the preset 20V limit.
In terms of electrical performance, implementation with a pre-regulator is similar to single-stage power conversion since the pre-regulator is only active during VBATT transients exceeding 20V. In addition, the total PCB area with the pre-regulator can still be smaller than a traditional implementation, which uses high-voltage discrete FETs and analog PWM controllers for single-stage conversion.
Figure 7 shows an implementation for a 12V lead-acid battery-powered automotive applications with two-stage power conversion. The first stage converts VBATT down to a 5V or 3.3V intermediate bus voltage. The second stage converts the intermediate bus voltage down to the SoC core rails using 6V-rated DrMOS devices.
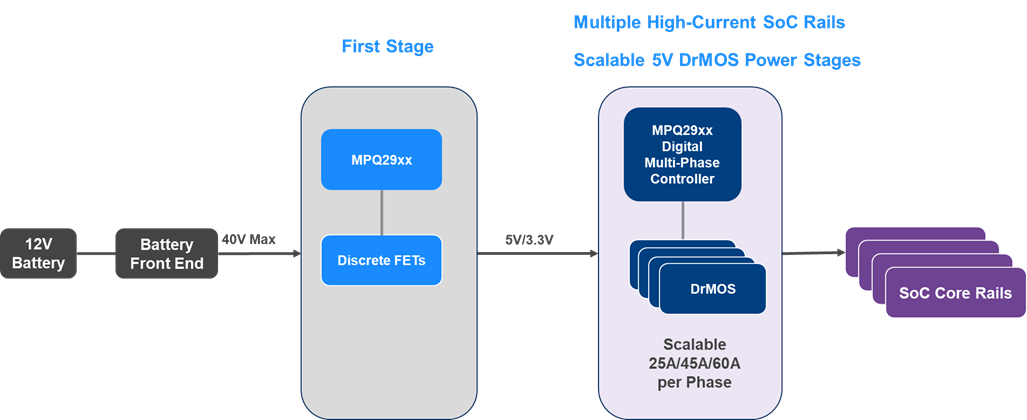
Figure 7: Two-Stage Power Conversion Using 6V-Rated DrMOS
Two-stage conversion requires additional semiconductor components, but the overall SoC power solution can be smaller and less expensive for low- to mid-power level SoC core rails when compared to a single-stage solution. The system designer must weigh all the factors (e.g. 12V battery chemistry and SoC core rail power specifications) to choose the optimal power architecture for their design.
Figure 8 shows a simplified application schematic using MPS’s digital, multi-phase controller and monolithic DrMOS power stages. The MPQ2977-AEC1 is configured for two output rails and three phases for each rail. This comprehensive solution requires fewer external components, while implementing several monitoring and protection features such as over-current protection (OCP), over-voltage protection (OVP), and over-temperature protection (OTP).
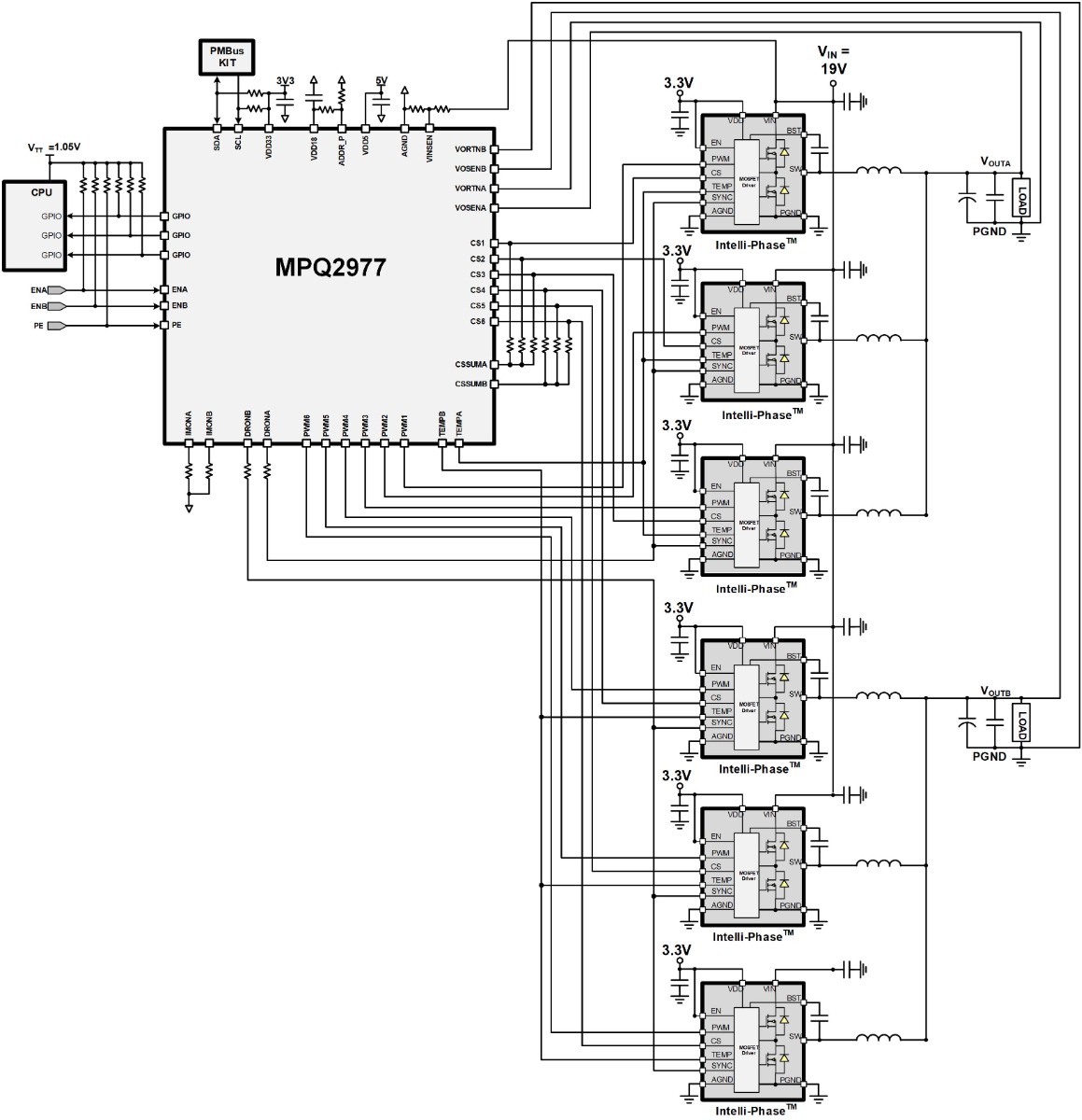
Figure 8: The MPQ2977
In addition, digital controllers are a part of MPS’s automotive MPSafeTM portfolio of safety-oriented, automotive-qualified products developed in accordance with the ISO 26262 Functional Safety Product Development Process. The ISO 26262 international standard has become a core requirement to address the risks of failure in electrical and electronic systems as the automotive industry pursues increasingly autonomous, connected, and electrified cars. The MPSafeTM product development process ensures that applicable automotive products can help customers meet various Automotive Safety Integrity Level (ASIL) requirements up to ASIL-D.
Conclusion
The automotive industry is undergoing a digital transformation towards autonomous driving, high-end infotainment systems, connectivity, and shared mobility to solve the problems faced by today’s drivers and passengers.
As a result, automotive computing architecture is evolving from a distributed architecture to a centralized architecture enabled by powerful SoCs. The SoCs used in central computing require advanced power management solutions, particularly for core voltage rails. Traditional power solutions are no longer well-suited for next-generation central compute power applications.
The state-of-the-art power management solutions, digital multi-phase controllers, and DrMOS power stages used in automotive SoC core power applications must deliver scalable, flexible, and compact power solutions with high efficiency and fast transient response. This article explained how to implement a power architecture with single-stage or two-stage power conversion. To learn more about MPS’s automotive SoC core power solutions, explore our AEC-Q100 grade, multi-phase digital controllers and DrMOS power stages.
_______________________
Did you find this interesting? Get valuable resources straight to your inbox - sent out once per month!
Log in to your account
Create New Account